What Are The Functions of Mitochondria: A Shocking Age Predictor
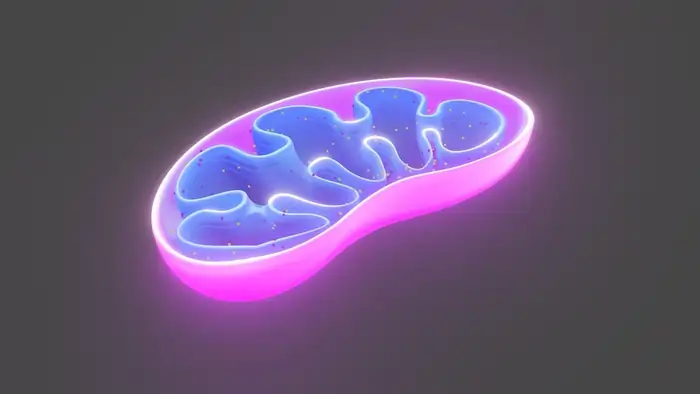
Mitochondria do more than serve as cellular energy factories. Their health directly affects how we age at the molecular level. The body shows various signs of aging when mitochondrial decline starts. Scientists consider mitochondrial dysfunction a key indicator to assess biological age. Mitochondrial decline happens when these vital organelles gradually break down. This breakdown speeds up aging and affects our skin’s appearance and brain function. This piece explores these remarkable cellular structures, their gradual decline over time, and ways we might slow or reverse their aging process.
What Are The Functions of Mitochondria in Human Cells
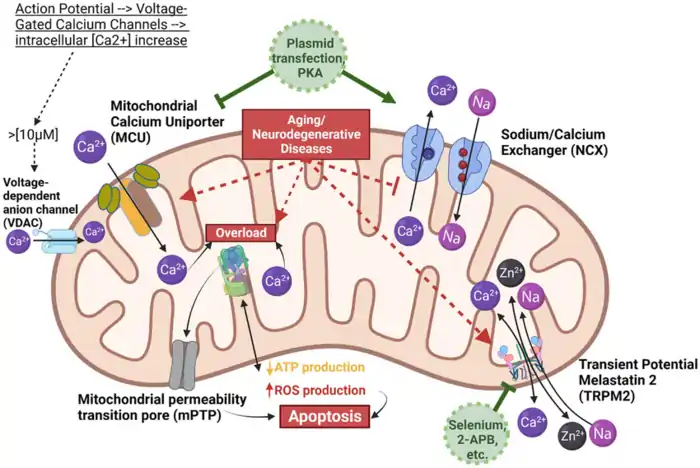
Image Source: ResearchGate
“Yet these rod-shaped organelles take on other roles as well, including serving as chemical factories and making key molecules necessary for building proteins and other cellular components.”
— Science staff writer (quoting research summary), Science Magazine reporting on mitochondrial research
Mitochondria work as sophisticated metabolic hubs in our cells. These remarkable organelles do much more than just produce energy. They contain specialized compartments where vital biochemical processes happen. Let me show you their main functions and how they connect to mitochondria and aging.
ATP Production via Oxidative Phosphorylation
What are the functions of Mitochondria at its most fundamental level? Its primary job is to produce adenosine triphosphate (ATP) through a process called oxidative phosphorylation (OXPHOS). This highly efficient energy conversion system creates about 30–32 ATP molecules from a single glucose molecule—far more than the 2 ATP produced by glycolysis.
The answer to what are the functions of Mitochondria continues with its role in managing the electron transport chain (ETC), which is embedded in the inner mitochondrial membrane. Here, reduced coenzymes NADH and FADH2 deliver high-energy electrons that drive the ATP-generating machinery. The chain has four protein complexes built into the inner membrane:
- Complex I (NADH dehydrogenase): Takes electrons from NADH
- Complex II (Succinate dehydrogenase): Takes electrons from FADH2
- Complex III (Cytochrome bc1): Moves electrons from reduced coenzyme Q to cytochrome c
- Complex IV (Cytochrome oxidase): Moves electrons to molecular oxygen
Complexes I, III, and IV pump protons (H+ ions) from the mitochondrial matrix into the intermembrane space as electrons flow [1]. This creates an electrochemical gradient across the inner membrane. The gradient includes a pH difference of about one unit and an electrical potential of 0.14 volts [1]. Each proton carries potential energy of about -5 kcal/mol [1].
ATP synthase (Complex V) uses this proton gradient through a fascinating mechanical process. Protons flowing back into the matrix through ATP synthase make its parts rotate. This rotation helps the enzyme create ATP from ADP and inorganic phosphate [1]. Each electron pair transfer pushes out about four protons through complexes I, III, and IV [1].
The whole oxidative phosphorylation process needs oxygen as its final electron acceptor. That’s why mitochondria use about 90% of the oxygen we breathe [1]. As we age, mitochondria often become less efficient at using oxygen and making ATP.
Calcium Homeostasis and Apoptosis Regulation
What are the functions of Mitochondria beyond producing energy? One critical function is controlling cellular calcium levels. The inner mitochondrial membrane contains specialized calcium transporters, including the mitochondrial calcium uniporter (MCU) and the mitochondrial Na⁺/Ca²⁺ exchanger (NCLX) [2][2]. These transporters maintain proper calcium balance within the organelle, which in turn affects numerous physiological processes.
Maintaining the right mitochondrial calcium levels activates key enzymes in the tricarboxylic acid (TCA) cycle, such as isocitrate dehydrogenase and α-ketoglutarate dehydrogenase [2]. This allows mitochondrial calcium to directly influence ATP production and balance energy demand within cells.
Another answer to what are the functions of Mitochondria is its role in programmed cell death (apoptosis). Mitochondria house various proteins that regulate the intrinsic apoptotic pathway, especially members of the Bcl-2 family [3][3]. Mitochondrial outer membrane permeabilization (MOMP) acts as a key checkpoint, triggering the caspase cascade that leads to cell death [3].
Pro-apoptotic proteins like Bax and Bak puncture the mitochondrial membrane during apoptosis, releasing cytochrome c into the cytosol [3]. Cytochrome c then binds to Apaf-1 and forms a complex with caspase-9 to initiate cellular destruction [3]. As we age, this regulatory balance often shifts toward increased apoptotic signaling.
Mitochondrial Role in Metabolic Signaling
Mitochondria do more than just make energy and control calcium. They coordinate several metabolic pathways that create important signaling molecules. The TCA cycle makes metabolites that work beyond their usual energy metabolism roles [4].
Acetyl-CoA, to name just one example, helps with protein lysine acetylation. This process changes metabolic enzymes and histones [4]. Citrate regulates central carbon metabolism by stopping glycolytic enzymes like phosphofructokinase and starting acetyl-CoA carboxylase for fatty acid synthesis [4].
Succinate buildup can stop 2-oxoglutarate-dependent dioxygenases (2OGDD), which changes genes and how they work [4]. Succinate even acts like a hormone by activating the succinate receptor GPR91, which has big effects on how our body works [4].
These metabolic signaling functions get worse as we age. This decline is a big part of what we call mitochondrial dysfunction aging. When these organelles work less well, they can’t keep up proper metabolic signaling. This speeds up cellular aging throughout the body.
How Mitochondrial Decline Begins with Age
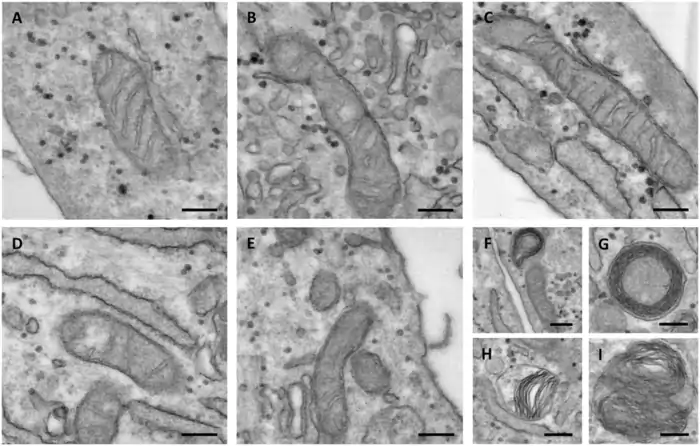
Image Source: ResearchGate
Mitochondrial health gets worse as we age – a basic process in cellular aging that starts earlier than most people think. The decline happens slowly through connected mechanisms that end up affecting the vital functions we discussed earlier.
What is Mitochondrial Decline?
What are the functions of Mitochondria as we age? Over time, mitochondrial decline becomes more evident. This natural process, along with certain diseases, leads to reduced ATP production, poorer management of oxidative stress, and significant changes in mitochondrial structure and function [5].
The mechanisms behind this decline are complex. Mitochondrial DNA (mtDNA) mutations accumulate due to oxidative damage, disrupting normal mitochondrial function and making ATP production less efficient [5]. Additionally, the cell’s quality control system, including mitophagy (which removes damaged mitochondria), also deteriorates [5]. As mitophagy breaks down, damaged mitochondria accumulate, further exacerbating cell dysfunction.
Age remains the primary risk factor for mitochondrial decline [5]. What are the functions of Mitochondria in this context? It produces less energy over time, leading to reduced ATP levels. This impacts almost every cellular process. The decline in mitochondrial function accelerates aging and contributes to various age-related diseases.
Age-Related Changes in Mitochondrial Morphology
Scientists can spot the first signs of mitochondrial aging by looking at their structure. Research with C. elegans shows that young animals have connected mitochondrial networks that break apart with age [6]. Muscle tissues also lose mitochondrial volume as they get older [7].
This breaking apart happens faster in conditions that shorten life and slower in ones that extend it [7]. But scientists found something interesting – they can’t predict how long five- and seven-day-old wild-type animals will live just by looking at their mitochondria’s shape or volume [7]. This tells us that while these changes mark aging, they result from aging rather than cause it.
Several molecules play key roles in these structural changes. DRP1 (dynamin-related protein 1) levels drop with age, which reduces mitochondrial splitting and movement [6]. Scientists discovered that in flies, the cyclic AMP/protein kinase A pathway controls mitochondrial transport, and PKA’s catalytic subunit decreases with age [6]. They can reverse this decline by adding cAMP or increasing PKA [6].
MFN2 (Mitofusin 2) protein levels also drop in aged muscle tissue, which matches the decrease in mitochondrial movement [6]. Mice without Mfn2 show that their axonal mitochondria pause more often and move slower in both directions [6].
Decreased mtDNA Copy Number and Enzyme Activity
Age brings molecular changes too. Studies show big drops in mtDNA copy numbers in skeletal muscles (23-40%) and liver (50%), but heart tissue stays the same [8]. People lose about 4 mtDNA copies every decade [9].
Different tissues react differently based on how much energy they use. Highly active tissues like red soleus muscle or liver can maintain their cytochrome c oxidase (COX) transcript levels even with less mtDNA [8]. Less active mixed fiber gastrocnemius muscle sees a 17-22% drop in transcript levels with age [8]. COX enzyme activity stays steady in aging liver and heart but drops 32% in lateral gastrocnemius muscle [8].
Human studies reveal that mtDNA heteroplasmy (having both normal and mutated mtDNA) increases with age while mtDNA copy numbers drop [9]. These changes happen independently rather than causing each other [9].
Somatic mtDNA Mutations and Their Role in Aging
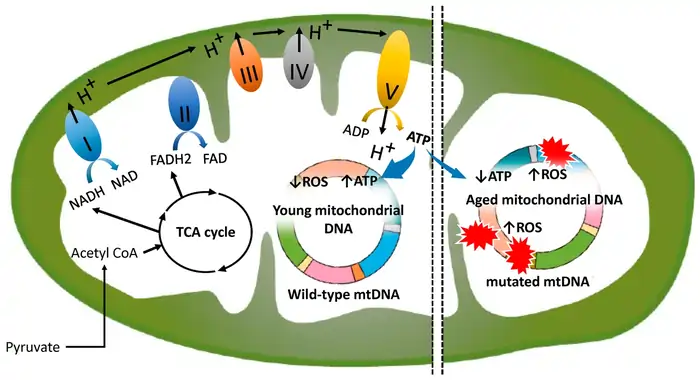
Image Source: MDPI
Somatic mutations in mitochondrial DNA are key pieces of the aging puzzle. Our cells divide throughout life, and small errors build up in mtDNA. These errors gradually weaken mitochondrial function and speed up biological aging. Scientists now understand how these mutations connect mitochondria and aging.
mtDNA Mutation Accumulation in Tissues
Different tissues show unique patterns in how mtDNA mutations build up. Postmitotic tissues like skeletal muscle, heart, and brain have higher levels of mtDNA deletions than fast-dividing tissues such as kidney, spleen, skin, and liver [1]. This distribution shows how mtDNA damage affects organs differently based on how often their cells divide.
These mutations don’t spread evenly through tissues but cluster in specific cells. COX-deficient skeletal muscle fibers in older people show very high levels (49%-94%) of clonally expanded mtDNA point mutations [1]. People over 65 often have point mutations in their skin fibroblasts and skeletal muscles. These mutations affect major regulatory regions for mtDNA replication in up to 50% of their cells [1].
The buildup of mutations speeds up over time. Scientists used advanced Duplex Sequencing to find more than 89,000 separate somatic mtDNA mutations in eight tissues of aged mice [10]. Each tissue showed different mutation rates in older mice. Kidney tissue had the highest SNV frequency (6.60±0.56 × 10–6) while heart tissue showed the lowest (1.74±0.16 × 10–6) [10].
Threshold Effects and Heteroplasmy
Heteroplasmy happens when normal and mutated mtDNA exist in the same cell. Unlike nuclear DNA, cells have multiple mtDNA copies, so mutated and wild-type versions can coexist. The amount of mutated mtDNA determines how much the cell malfunctions through “threshold effects”:
- Phenotypic threshold effect: Symptoms appear only after mutant mtDNA reaches 60-80% [11]
- Biochemical threshold effect: Respiratory chain complex activity can drop without affecting mitochondrial respiration or ATP synthesis until it hits a critical point [2]
- Translational threshold effect: MtDNA mutations must reach certain levels to affect mitochondrial protein synthesis [2]
These thresholds explain why similar mutations can cause very different clinical symptoms. They also show why many people with potentially harmful mutations stay healthy—their mutation levels stay low enough for normal mtDNA to compensate [12].
mtDNA Mutator Mouse Model Insights
The mtDNA mutator mouse has changed how we understand mitochondrial aging. These mice have a proofreading-deficient mtDNA polymerase γ (POLG), which makes mtDNA mutations accumulate faster [13]. As a result, they age prematurely, with shorter lives, heart problems, and muscle loss [13].
This model reveals interesting patterns in how mutations spread through mitochondrial DNA. Next-generation sequencing shows point mutations distribute fairly evenly across the mitochondrial genome [13]. Mutator mice typically get about 20 mutations per mtDNA molecule [1]. Each COXI gene has an 88% chance of at least one mutation and a 61% chance of two or more—40% of these change amino acids [1].
Scientists still debate whether point mutations or deletions matter more. Loeb’s team found only increased point mutations in COX-negative cells, with no extra deletions [1]. Other studies suggest mutator mice get one deletion for every 1,000 point mutations [1]. This ratio hints that point mutations likely cause the aging symptoms in these mice.
Reactive Oxygen Species and the Vicious Cycle of Damage
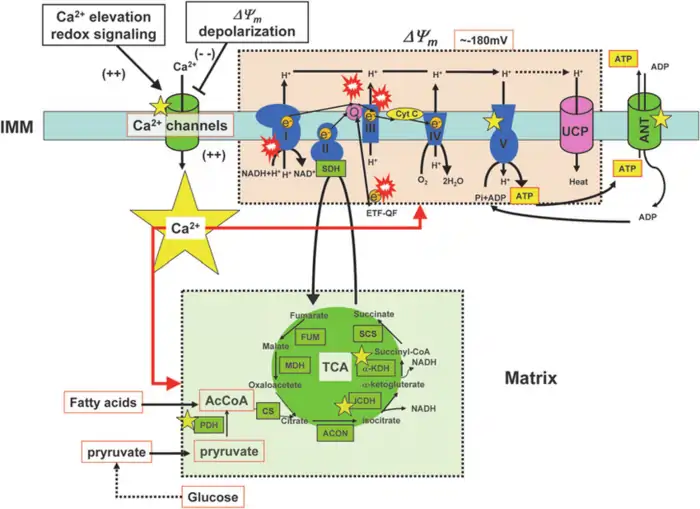
Image Source: ResearchGate
“It has been established that mitochondrial respiratory function declines with age, and that defects in the respiratory chain increase the production of ROS and free radicals in mitochondria.”
— Guido Kroemer, Cell biologist, author of the cited PubMed article
The generation of reactive oxygen species (ROS) in mitochondria creates a dangerous feedback loop that speeds up aging. This mechanism shows one of the most basic processes in mitochondrial dysfunction aging. Knowing how ROS damages mitochondria explains why these organelles can predict biological age reliably.
ROS Generation at Complex I and III
What are the functions of Mitochondria in the context of reactive oxygen species (ROS)? It produces about 90% of all ROS in the body [14], acting as the cell’s primary source of oxidative byproducts. During normal oxidative metabolism, cells convert approximately 1–5% of consumed oxygen into ROS instead of water [15]. This occurs at key sites within the electron transport chain.
Complex I (NADH dehydrogenase) releases superoxide directly into the mitochondrial matrix, where mitochondrial DNA (mtDNA) resides [3]. It generates ROS via both forward electron transfer and reverse electron transport (RET) [16]. RET, which occurs when electrons flow backward from Complex II through ubiquinone to Complex I, is especially prone to high ROS output under conditions of elevated membrane potential [17].
Complex III (ubiquinone-cytochrome c reductase) is unique in producing superoxide on both sides of the inner mitochondrial membrane—into the matrix and the intermembrane space [3]. This dual output makes Complex III’s ROS more accessible to the cytosol for signaling purposes. Under normal metabolic conditions, Complex III is considered the primary source of mitochondrial ROS [15].
Oxidative Stress and Mitochondrial Feedback Loop
What are the functions of Mitochondria as it loses functional integrity? One major consequence is that it begins converting a larger portion of oxygen into reactive oxygen species (ROS), setting off a destructive cycle [15]. Normally, only about 0.2–2% of oxygen molecules are converted into superoxide anions [4], but this percentage rises as mitochondrial efficiency declines.
This cycle begins when ROS damages key components of the electron transport chain—particularly Complexes I and III. Damaged complexes transport electrons less efficiently, causing increased electron leakage and even more ROS production [16]. This self-reinforcing loop of oxidative stress and mitochondrial breakdown lies at the core of the mitochondrial theory of aging.Mitochondrial calcium overload often triggered by oxidative stress makes the mitochondrial permeability transition pores (mPTP) open [16]. This guides to:
- Loss of cytochrome c from the inner mitochondrial membrane
- Impeded electron transport between complexes
- Further increases in electron leakage and ROS generation
Oxidative stress triggers events that create even more oxidative stress. This positive feedback loop compromises ATP production, damages mitochondrial membranes, and activates apoptotic pathways [18].
ROS-Induced mtDNA Damage
The mitochondrial genome is especially vulnerable to oxidative damage for several reasons. MtDNA is located right next to the inner mitochondrial membrane and the electron transport chain—precisely where reactive oxygen species (ROS) are produced [17]. Unlike nuclear DNA, it lacks protective histone proteins [17], and mitochondria have less comprehensive DNA repair systems than the nucleus [19].
These vulnerabilities make mtDNA about ten times more likely to suffer oxidative damage compared to nuclear DNA [17]. Experiments show that when cells are treated with hydrogen peroxide (H₂O₂), mitochondrial DNA incurs more extensive and longer-lasting damage than nuclear DNA [20]. While nuclear DNA typically repairs itself within 1.5 hours, mtDNA often remains impaired for much longer [20].
So, what are the functions of Mitochondria when this damage builds up? It struggles to produce energy efficiently. Critical bioenergetic genes accumulate mutations or deletions that disrupt oxidative phosphorylation [17]. This leads to further energy instability and accelerates mitochondrial decline and cellular aging. This vicious cycle is central to the mitochondrial theory of aging: ROS-induced mtDNA damage causes a progressive loss of function, creating a self-perpetuating loop of decline [19].
Mitochondrial Dynamics: Fission, Fusion, and Quality Control
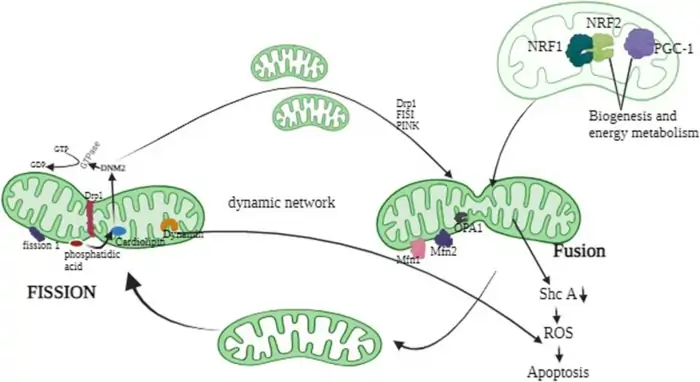
Image Source: ResearchGate
Mitochondria need to split and merge constantly to stay healthy at the cellular level. This process of fission and fusion keeps mitochondrial quality in check. Scientists now know these dynamic processes play a central role in mitochondria and aging mechanisms.
DRP1 and MFN2 in Aging Muscle and Brain
Dynamin-Related Protein 1 (DRP1) plays a vital role in mitochondrial fission. So, what are the functions of Mitochondria with DRP1? It recruits DRP1 to the outer membrane, where DRP1 forms a collar-like structure and constricts the mitochondria to divide them. DRP1 behavior shifts in different tissues as we age. Exercise training significantly raises DRP1 levels in the cortex of older mice—an effect not seen in younger mice [21]. These elevated levels may help reverse age-related brain changes by improving mitochondrial delivery to synapses and reducing oxidative stress, ultimately supporting neuronal survival [21].
The story is different in aging muscle. Studies show that DRP1 levels decline with age in human skeletal muscle [5], disrupting the balance between fission and fusion. Although scientists continue to debate DRP1’s full range of functions, its importance is clear. Reducing or removing DRP1 in skeletal muscle leads to 40–50% muscle atrophy [22], highlighting its essential role in preserving muscle health.
Mitofusin 2 (MFN2), which fuses the outer mitochondrial membranes, also changes with age. New findings suggest that extra MFN2 can promote mild muscle growth and may help offset age-related muscle decline [5]. However, results across studies are inconsistent—some report decreased levels, others show increases, and some find no significant change [22]. These discrepancies likely reflect tissue-specific differences and varying research methods.
OPA1 and Mitochondrial Network Remodeling
Optic Atrophy 1 (OPA1) serves two important purposes within the mitochondria. What are the functions of Mitochondria with OPA1? It helps fuse inner membranes and maintains the cristae structure independently. OPA1 exists in two forms: long membrane-anchored (L-OPA1), which enables fusion, and short soluble form (S-OPA1), which cannot [23]. The balance between these two forms is crucial in shaping mitochondrial structure.
Research shows mixed results about OPA1’s aging effects. Some studies found less OPA1 content in inactive elderly people’s muscles compared to complex II levels, but not in active seniors [22]. Other research indicated OPA1 decreased in both high and low functioning elderly [22]. Just one week of exercise brought OPA1 levels back up in aged skeletal muscles [22], highlighting potential strategies for mitochondrial health.
OPA1’s role in quality control within the mitochondria deserves a closer look. It keeps mitochondrial DNA stable, preventing mitochondrial network fragmentation and mtDNA loss in cell models [24]. OPA1 also maintains cristae structure, which helps respiratory chain supercomplexes stay stable [6], making metabolism more efficient.
What Imbalanced Dynamics Mean for Cell Health
Cells start breaking down when mitochondrial fission-fusion balance fails. This speeds up mitochondrial dysfunction aging. Problems include:
- Less ATP production and worse bioenergetics
- Disrupted calcium balance affecting cell signals
- Poor cleanup of damaged mitochondria
- Changed cell death signals through cristae reshaping
The link between mitochondrial dynamics and quality control is especially evident in brain diseases. In Alzheimer’s disease, beta-amyloid plaques reduce mitochondrial fusion by interfering with MFN1/2, while simultaneously increasing fission through elevated levels of DRP1 and FIS1 [25]. In Parkinson’s disease, the buildup of α-synuclein and problems with the PINK1/PARKIN pathway alter mitochondrial fragmentation [25].
Scientists continue to debate the exact role of mitochondrial dynamics in aging. However, research suggests that it’s the balance between fission and fusion—not an excess of either—that helps cells stay healthy. Exercise appears to positively influence this balance [22], which hints at potential ways to improve mitochondrial health as we age.
What are the functions of Mitochondria in terms of cellular health? The balance of dynamics within mitochondria plays a crucial role in maintaining cell vitality and is a critical factor in predicting biological aging.
Mitophagy and Biogenesis: The Balancing Act
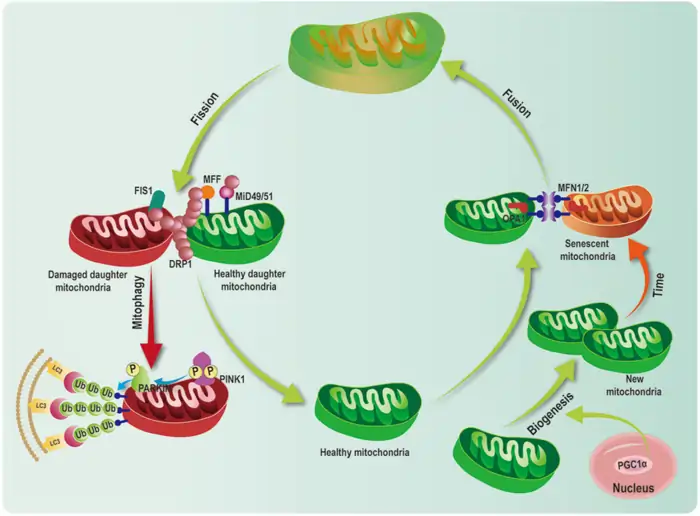
Image Source: ResearchGate
A delicate balance between removing damaged organelles and creating new ones keeps mitochondrial health in check. This renewal process slows down as we age and leads to mitochondria aging and poor function.
PINK1/Parkin Pathway in Mitophagy
The PINK1/Parkin pathway acts as the main quality control system for damaged mitochondria. Damaged mitochondria lose their membrane potential, which causes PINK1 (PTEN-induced kinase 1) to build up on the outer membrane rather than being imported and broken down [7]. PINK1 then adds phosphate to ubiquitin at Ser65. This creates a signal that pulls Parkin from the cytosol [26]. The activated Parkin makes this signal stronger by adding ubiquitin chains to proteins on the mitochondrial outer membrane [7].
These ubiquitin additions attract autophagy adaptors like NDP52 and Optineurin. They connect damaged mitochondria to autophagosomes that break them down [27]. The pathway becomes less effective with age, and damaged mitochondria start to pile up. This speeds up mitochondrial decline.
PGC1α and NRF1 in Mitochondrial Biogenesis
New mitochondria must replace the damaged ones removed through mitophagy. What are the functions of Mitochondria in this process? Mitochondria rely on a precise system for renewal, with PGC-1α leading the replacement process [28]. AMPK phosphorylation or SIRT1 deacetylation activates PGC-1α, which then triggers various nuclear transcription factors, including NRF-1 and NRF-2 [28].
PGC-1α works through NRF-1/2 to boost the production of mitochondrial transcription factor A (TFAM). TFAM directly increases mtDNA transcription and replication [9]. Studies in animals show that higher levels of PGC-1α lead to the creation of more mitochondria, which enhances energy production and metabolic rate [29].
Coordination Between Turnover and Synthesis
Healthy mitochondria require a delicate balance between mitophagy and biogenesis. Research shows that these processes share control mechanisms. For instance, exercise triggers both Nrf2 and PGC-1α, which play vital roles in mitochondrial biogenesis [8].
What are the functions of Mitochondria in this context? PGC-1α surprisingly impacts both sides of mitochondrial health—it stimulates both the creation and removal of mitochondria. It can trigger mitophagy by interacting with mitostatin [28], acting as a coordinator between the removal and creation cycles of mitochondria.
Poor regulation of these processes is a significant factor in what is known as mitochondrial decline. As we age, mitophagy becomes less efficient, allowing damaged mitochondria to accumulate. At the same time, reduced biogenesis limits the creation of new mitochondria, leading to a gradual shift toward fewer and lower-quality mitochondria—an essential sign of mitochondrial dysfunction and aging.
How Mitochondria Predict Your Biological Age
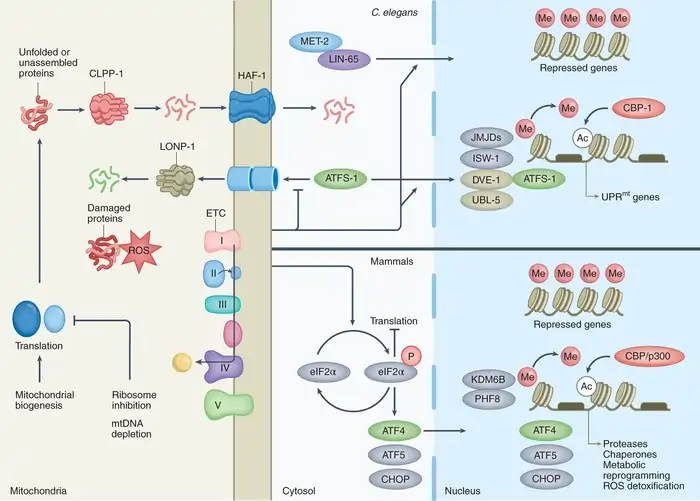
Image Source: Nature
Scientists now look at mitochondria to determine our true biological age, which differs by a lot from our actual years. New research shows how these cellular powerhouses help us understand how our bodies age at the molecular level.
Mitochondrial Health as a Biomarker
The link between mitochondrial function and aging goes beyond theory and provides measurable markers of biological aging. What are the functions of Mitochondria in this case? Mitochondria, through their DNA, play a key role in cellular health and aging. Mitochondrial DNA copy number (mtDNAcn) measures the abundance of mitochondrial genomes, offering insights into both oxidative stress levels and overall mitochondrial function [30]. This number typically declines with age and is positively associated with telomere length [30].
A study involving 812 aging male veterans revealed something surprising: while mtDNAcn showed negative correlations with DNAm-Age and DNAm-PhenoAge at any given time, higher mtDNAcn actually predicted faster biological aging, regardless of chronological age [30]. This suggests that what the mitochondria do is actively involved in the aging process, not merely responding to it.
mtDNA Deletion Load and Aging Clock
Mitochondrial DNA deletions create another powerful way to measure aging. Unlike point mutations, these deletions cluster in specific tissues and grow within cells. The common mtDNA4977 deletion in human brain tissue shows dramatic age-related increases. It jumps from 0.16%-1% in people aged 67-77 to about 12% in those over 80 [31]. These deletions also increase exponentially with age in human skeletal muscle [32]. This is a big deal as it means that Alzheimer’s patients have 15 times more mtDNA deletions across multiple brain regions compared to others their age [33]. Parkinson’s patients’ neurons show up to 52% deleted mtDNA versus 43% in age-matched controls [33].
Mitochondrial Function Tests in Clinical Use
Doctors now use mitochondrial function tests to determine biological age. But what are the functions of Mitochondria in this context? Recent research shows that mitochondrial function can predict overall health and aging. For example, the largest longitudinal study of over 600 older adults by Johns Hopkins researchers found that free-floating mitochondrial DNA fragments in blood samples are linked to chronic inflammation and frailty [34]. These DNA fragments, which come from dying cells, accurately predict cognitive and physical decline [34].
Non-invasive measurements of phosphocreatine (PCr) recovery time offer a real-time assessment of mitochondrial respiratory capacity [35]. When combined with other measures such as mtDNA heteroplasmy levels and haplogroup analysis, doctors can now build comprehensive profiles of mitochondrial health. These profiles help predict overall health status more effectively than age alone [36].
Can You Reverse Mitochondrial Aging?
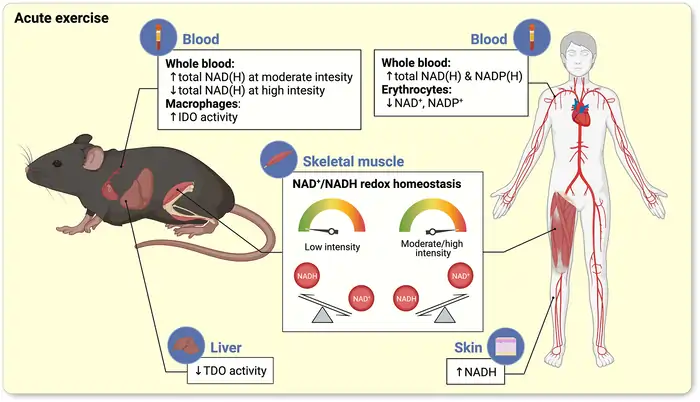
Image Source: Wiley Online Library
Scientists have found that there are promising ways to reverse mitochondrial deterioration that could turn back your biological clock. The most important approaches involve your body’s natural renewal systems.
Caloric Restriction and Exercise Effects
Caloric restriction without malnutrition shows powerful mitochondrial benefits. Research shows CR preserves mitochondrial function by protecting existing components rather than increasing biogenesis [37]. This protection happens through decreased mitochondrial ROS production and less oxidative damage to DNA and proteins [37]. A 6-month low-calorie diet showed improved metabolism by boosting mitochondrial dynamics and reducing ROS in humans [38].
Exercise is one of the most available ways to improve mitochondrial health. Regular physical activity helps mitochondria adapt through several ways:
- It increases expression of fusion proteins (OPA1, DRP1, MFN2) as shown in a 12-week swimming study [10]
- It boosts expression of PGC-1α and other biogenesis markers [10]
- It improves mitochondrial protein synthesis and quality control [10]
Just one intense aerobic workout can lead to lasting increases in mitochondrial proteins [10].
NAD+ Boosters and Sirtuin Activation
NAD+ levels drop significantly with age, which negatively impacts sirtuin activity. What are the functions of Mitochondria in relation to this? Mitochondria rely on NAD+ for various functions, and the decline in NAD+ levels affects their efficiency. Research suggests that restoring NAD+ through precursors like nicotinamide mononucleotide (NMN) or nicotinamide riboside (NR) could help reverse some of these effects [39]. Studies with mice have shown that NMN supplements improve endothelial dysfunction [40] and reduce heart attack damage [40].
Sirtuin-activating compounds (STACs) offer another potential solution. Resveratrol, the first STAC discovered, protects against the detrimental effects of high-calorie diets, slows age-related diseases, and helps mice exercise longer [41]. Newer STACs have been developed that work even better and more specifically, offering a promising avenue for improving mitochondrial function [42].
Senolytics and Mitophagy Inducers
Aging cells collect dysfunctional mitochondria that don’t produce energy efficiently [43]. Senolytic compounds that remove aging cells could help solve this problem. Natural compounds like fisetin and quercetin can clear out defective cells that build up with age [2].
On top of that, mitophagy inducers help remove damaged mitochondria. PARP inhibitors like AZD2281 helped worms live 15-23% longer by improving mitophagy [39]. The resulting increase in NAD+ levels activated sirtuins, which led to better mitochondrial breathing and energy production [39].
Conclusion
Mitochondrial biology reveals a striking truth – these cellular powerhouses paint a clearer picture of our aging process than our actual years. Scientists can measure our true biological age by looking at our mitochondria’s health markers like mtDNA copy number, deletion load, and functional capacity. These tiny powerhouses show signs of aging at multiple levels as ATP production drops and quality control systems start failing.
Mitochondria’s complex connection to aging highlights why their health is crucial to living longer. Scientists have found that mtDNA mutations build up while dynamics get thrown off balance. This creates a destructive loop where oxidative damage speeds up how fast our cells age. The good news is that we can break this cycle with the right approach.
Research suggests we can turn back mitochondrial aging through lifestyle changes and new treatments. A carefully restricted diet protects mitochondrial parts and cuts down harmful ROS production. Regular exercise makes quality control systems work better and gets more mitochondria growing through PGC-1α pathways. On top of that, substances that increase NAD+ levels or activate sirtuins could help restore mitochondria’s function, even in older adults.
Scientists now see mitochondria as key players in aging rather than passive bystanders. These cellular components shape our biological timeline and maybe serve as the best predictor of our body’s decline rate. Learning about these processes gives us amazing chances to influence aging at its core – right inside the cellular powerhouses that have kept life going since its earliest days.
FAQs
How do mitochondria contribute to the aging process?
Mitochondria play a crucial role in aging by regulating various metabolic and signaling pathways. As we get older, mitochondrial function declines, leading to decreased energy production, increased oxidative stress, and compromised cellular processes. This decline contributes to age-related diseases and overall biological aging.
What are the functions of mitochondria in our cells?
Mitochondria are primarily responsible for producing energy through a process called oxidative phosphorylation. They also regulate cell growth and death, store calcium, and participate in cell signaling. Additionally, mitochondria contain their own DNA and are involved in various cellular processes that affect overall health and aging.
Can mitochondrial health predict biological age?
Yes, mitochondrial health can be a strong indicator of biological age. Markers such as mitochondrial DNA copy number, deletion load, and functional capacity offer insights into how our bodies are aging at the cellular level. These markers often provide a more accurate picture of biological age than chronological age alone.
How do lifestyle factors affect mitochondrial health?
Lifestyle factors significantly impact mitochondrial health. Regular exercise promotes mitochondrial adaptations by increasing the expression of important proteins and enhancing mitochondrial quality control. Caloric restriction without malnutrition has been shown to protect existing mitochondrial components and reduce harmful reactive oxygen species production.
Are there ways to reverse mitochondrial aging?
Emerging research suggests that mitochondrial aging may be partially reversible. Strategies include lifestyle interventions like exercise and caloric restriction, as well as targeted therapies such as NAD+ boosters and sirtuin activators. These approaches aim to enhance mitochondrial function, promote quality control mechanisms, and potentially slow down or reverse aspects of cellular aging.
References
[1] – https://pmc.ncbi.nlm.nih.gov/articles/PMC2815752/
[2] – https://pmc.ncbi.nlm.nih.gov/articles/PMC9784569/
[3] – https://pmc.ncbi.nlm.nih.gov/articles/PMC2933303/
[4] – https://www.frontiersin.org/journals/physiology/articles/10.3389/fphys.2021.627837/full
[5] – https://onlinelibrary.wiley.com/doi/full/10.1111/acel.70054
[6] – https://pmc.ncbi.nlm.nih.gov/articles/PMC4457892/
[7] – https://www.nature.com/articles/s41392-023-01503-7
[8] – https://pmc.ncbi.nlm.nih.gov/articles/PMC6527603/
[9] – https://www.nature.com/articles/s41467-022-28247-2
[10] – https://www.ahajournals.org/doi/10.1161/JAHA.124.036555
[11] – https://pmc.ncbi.nlm.nih.gov/articles/PMC8307225/
[12] – https://www.pnas.org/doi/10.1073/pnas.1403521111
[13] – https://pmc.ncbi.nlm.nih.gov/articles/PMC3335313/
[14] – https://pmc.ncbi.nlm.nih.gov/articles/PMC6942696/
[15] – https://pmc.ncbi.nlm.nih.gov/articles/PMC4145906/
[16] – https://www.mdpi.com/2076-3921/12/4/934
[17] – https://pmc.ncbi.nlm.nih.gov/articles/PMC8707347/
[18] – https://www.sciencedirect.com/science/article/abs/pii/S1568163725000133
[19] – https://academic.oup.com/nar/article/37/8/2539/2409966
[20] – https://www.pnas.org/doi/10.1073/pnas.94.2.514
[21] – https://pmc.ncbi.nlm.nih.gov/articles/PMC5346470/
[22] – https://pmc.ncbi.nlm.nih.gov/articles/PMC8348122/
[23] – https://www.frontiersin.org/journals/cell-and-developmental-biology/articles/10.3389/fcell.2021.626117/full
[24] – https://www.nature.com/articles/s41419-019-1953-y
[25] – https://www.mdpi.com/1422-0067/25/23/12855
[26] – https://pmc.ncbi.nlm.nih.gov/articles/PMC4764997/
[27] – https://actaneurocomms.biomedcentral.com/articles/10.1186/s40478-020-01062-w
[28] – https://pmc.ncbi.nlm.nih.gov/articles/PMC10215733/
[29] – https://journals.biologists.com/jcs/article/125/21/4963/32544/PGC1-and-mitochondrial-metabolism-emerging
[30] – https://pmc.ncbi.nlm.nih.gov/articles/PMC7041780/
[31] – https://www.frontiersin.org/journals/aging/articles/10.3389/fragi.2024.1359638/full
[32] – https://www.nature.com/articles/s43587-022-00191-2
[33] – https://pmc.ncbi.nlm.nih.gov/articles/PMC10902006/
[34] – https://www.hopkinsmedicine.org/news/newsroom/news-releases/2023/06/new-findings-show-mitochondrial-dna-fragments-in-blood-as-important-biomarkers-for-aging-and-inflammation
[35] – https://pmc.ncbi.nlm.nih.gov/articles/PMC4779179/
[36] – https://www.sciencedirect.com/science/article/pii/S0925443918304502
[37] – https://pubmed.ncbi.nlm.nih.gov/23217257/
[38] – https://www.sciencedirect.com/science/article/pii/S0891584924009973
[39] – https://pmc.ncbi.nlm.nih.gov/articles/PMC3753670/
[40] – https://www.frontiersin.org/journals/physiology/articles/10.3389/fphys.2021.755060/full
[41] – https://pmc.ncbi.nlm.nih.gov/articles/PMC5107309/
[42] – https://www.ahajournals.org/doi/10.1161/CIRCRESAHA.118.312498
[43] – https://www.sciencedirect.com/science/article/pii/S2352396417301160