Cellular Senescence and Aging: What Powerful New Research Reveals
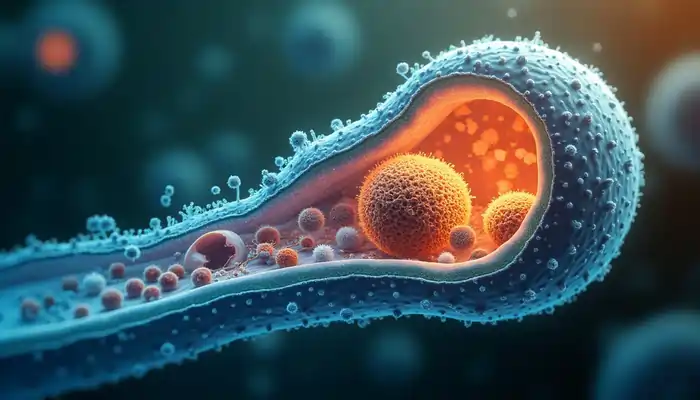
Our cells have built-in biological clocks that control how and when they age. Scientists have made amazing discoveries about the molecular mechanisms behind this aging process. The sort of thing I love is how these tiny cellular timekeepers work.
Understanding cellular senescence and the complex pathways of cell aging is a vital part of modern medicine. Scientists now explore deeply into these mechanisms and find how cells change from healthy, vibrant units to aged or dying ones. This knowledge goes beyond theory – it creates new possibilities to slow or maybe even reverse parts of cellular aging.
This piece takes you through the hidden world of molecular mechanisms of cellular aging, from their early-life vitality through mid-life changes to late-life breakdown. You’ll learn about advanced research methods and current limitations in anti-aging strategies. The way cellular aging impacts our overall health and longevity becomes clearer as we understand these processes better.
Molecular mechanisms of cellular aging: Foundations of Longevity
Cell health starts at the earliest stages of life. Most adult tissue cells age over time, but some cell groups show amazing resistance to aging.
High Telomerase Activity in Embryonic and Germline Cells
Life begins with cells that have powerful anti-aging mechanisms. These cells keep high levels of telomerase, an enzyme that adds protective telomeric repeats to chromosome ends. Scientists have found substantial telomerase activity in human blastocysts and developing tissues 16-20 weeks after fertilization [1]. This activity drops sharply after birth and becomes almost undetectable in most somatic tissues during the neonatal period.
Some cell populations still maintain their telomerase activity. Embryonic stem cells, germ cells in testes and ovaries, and certain stem cell populations keep this vital enzyme throughout life [2]. Nature preserves these cells to maintain telomere length and ensure genetic stability across generations.
Telomerase activation follows a precise timeline during early development. Research confirms that telomere elongation happens during the morula-to-blastocyst transition through telomerase-dependent mechanisms [3]. High telomerase activity in embryonic stem cells boosts their self-renewal abilities, growth potential, and differentiation efficiency [4].
Efficient DNA Repair and Low ROS in Youthful Cells
Young cells excel at DNA repair beyond just maintaining telomeres. Recent studies of single-strand annealing (SSA), a key DNA repair pathway, show young cells repair DNA with 93.8% efficiency. Older cells manage only 71.8% – a substantial 23.4% drop [5]. This repair ability helps young cells protect their genetic material from environmental damage.
The life-blood of early-cell health lies in redox homeostasis – the balance between reactive oxygen species (ROS) production and antioxidant defenses. Young cells stay healthier because:
Their mitochondria work more efficiently with fewer harmful byproducts
They have stronger antioxidant defense systems
Their cell cycle timing optimizes DNA repair
ROS serves two purposes in cell health. Too much ROS damages DNA, proteins, and lipids, but moderate levels work as vital signaling molecules [6]. Young cells master this balance, letting ROS send signals without causing damage.
Young cells also spend less time in the G1 phase compared to older cells [5]. Shorter G1 phases lead to better DNA repair since certain repair mechanisms work best during other cell cycle phases.
These basic mechanisms – high telomerase activity, quick DNA repair, and perfect redox balance – create the impressive resilience we see in young cells. These protective systems gradually decline as we age, showing up as cellular aging and age-related diseases.
Mid-Life Molecular Shifts: The Onset of Cellular Senescence
Our cells experience major molecular changes as we age through mid-life. These changes mark a crucial point where our cellular health moves from youthful resilience toward the beginning signs of decline.
Decline in NAD+ Levels and Sirtuin Activity
NAD+ levels represent one of the most important mid-life cellular changes. This critical metabolite powers many biological processes. Studies show NAD+ levels drop by 10-50% in aged liver tissue [7]. Human skin samples reveal an even more dramatic reduction—at least 50% throughout adult aging [8].
The reduction in NAD+ creates ripple effects throughout the body because it directly affects sirtuin activity. Sirtuins need NAD+ to function as enzymes that control many cellular processes including DNA repair, metabolism, and stress responses. Lower NAD+ levels reduce sirtuin function, which affects:
Communication between the nucleus and mitochondria [7]
Systemic communication between the hypothalamus and adipose tissue [7]
Circadian rhythm regulation and sleep quality [9]
Age-related inflammation activates CD38, and DNA damage triggers PARPs. These NAD+-consuming enzymes cause NAD+ levels to drop with age. Wild-type mice at 32 months typically have only half the NAD+ levels of young mice [10].
Accumulation of DNA Damage and Epigenetic Drift
DNA damage builds up in our cells as repair efficiency decreases. Young cells can repair certain types of damage with 93.8% efficiency. This drops to 71.8% in older cells—a substantial 23.4% decrease [11]. The accumulation triggers the DNA damage response (DDR), which can push cells toward senescence.
Epigenetic drift occurs across most of the genome as DNA methylation patterns change gradually. Global hypomethylation increases with age, yet certain genes become hypermethylated at their CpG islands. A meta-analysis revealed consistent hypermethylation patterns across 59 different tissues from 128 mammalian species [12].
Scientists have developed “epigenetic clocks” based on DNA methylation levels because these changes associate with age so reliably [12]. Changed methylation that reduces pentose metabolism genes seems to play a crucial role in cellular aging [12].
Early Signs of Cellular Senescence and SASP Activation
DNA damage and epigenetic alterations lead many cells into senescence—a permanent cell cycle arrest. Senescent cells show several unique features:
Upregulation of cyclin-dependent kinase inhibitors p16INK4a and p21CIP1 [13]
Persistent DNA damage response signaling [14]
Reduced lamin B1 expression and nuclear envelope disruption [14]
Increased senescence-associated β-galactosidase activity [14]
Senescent cells develop the senescence-associated secretory phenotype (SASP), maybe even their most defining characteristic. SASP includes cytokines, chemokines, growth factors, and proteases [15]. Interleukin-6 (IL-6), IL-1α, IL-1β, IL-8, and various IGF-binding proteins make up key SASP components [16].
Senescent cells influence their surroundings through these secretions. Scientists call SASP “the cause and consequence of inflammaging” [17]—the chronic, low-grade inflammation common in aging tissues.
All but one of these senescent cells make up less than 20% of total cells in aged tissues, yet their influence extends way beyond the reach and influence of their numbers [18]. Their inflammatory secretions can trigger senescence in nearby cells and magnify tissue damage through paracrine action [18].
Late-Life Cellular Breakdown: How Aging Becomes Pathology
Cells break down as we age. Natural aging turns into disease states because molecular damage piles up over decades. These changes show up as major functional problems in our later years.
Mitochondrial Dysfunction and ATP Depletion
Aging cells face an energy crisis that starts with substantial mitochondrial problems. Research shows liver mitochondria in old rats (24 months) work 40% less efficiently than in young ones (3-4 months) [19]. The decline shows several patterns:
ATP production becomes less efficient
Mitochondrial membranes lose their charge
ROS production goes into overdrive
Cells move from mitochondrial breathing to sugar burning for energy
The cell tries to fix this energy shortage by making more mitochondria, but these new ones don’t work well [20]. On top of that, it turns out mitochondria in old cells look different – they’re longer, bigger, and stuck together because they can’t split apart properly anymore [21].
This breathing problem causes NAD+ to drop by half in aged tissues [20]. The situation gets worse as low ATP levels turn on AMPK signals, which lock cells into senescence through p53 [4].
Autophagy Blockade and Protein Aggregate Accumulation
Our cells need to clean out damaged proteins and parts through autophagy. This cleanup system stops working as we get older. Broken proteins start piling up into what scientists call “age-ggregates” [3].
Studies show old samples have 1.3 to 2.5 times more stuck-together proteins than young ones [22]. These protein clumps are tough – they:
Won’t dissolve in detergents
Resist enzyme breakdown
Light up with amyloid-detecting dyes
Pile up in cellular trash heaps called aggresomes [3]
The protein quality control system falls apart completely. The cell’s cleanup crew – its proteasomes and chaperones – starts to malfunction [3]. These protein piles relate directly to how slowly cells grow [23].
Stem Cell Pool Depletion and Tissue Repair Failure
The body’s repair system takes a big hit when stem cells start dying off. Neural stem cells in the brain’s subventricular zone and hippocampus drop substantially with age. This drop matches the loss of smell and thinking abilities [24].
Stem cells fail in several ways. They lose their ability to make copies of themselves, as shown by lab tests and living tissue studies [24]. Many stem cells also get old and stop working, turning on blocking signals like p53/p21 and p16INK4a [25].
The body can’t fix itself as well anymore. Wounds heal slower in older skin because inflammation lasts too long and ROS levels spike [2]. Poor blood flow makes things worse – fewer repair cells reach the damaged areas [2]. Wounds that won’t heal are a huge problem, especially in older people who have other health issues [26].
Materials and Methods: How Aging is Studied at the Molecular Level
Modern molecular technologies give us new ways to understand how we age. Scientists use innovative technology to monitor cellular aging precisely. This has revolutionized our knowledge of cellular senescence.
Single-Cell Transcriptomics in Aging Research
Scientists now analyze gene expression in individual cells through single-cell RNA sequencing (scRNA-seq). This method has changed aging research completely. Traditional “bulk” methods average measurements across many cells, but scRNA-seq eliminates cell composition bias [1]. Scientists created single-cell transcriptomic clocks that could predict human blood cell age accurately. The mean absolute errors ranged between 8.64 and 10.48 years [1].
These techniques showed a strong link between transcriptomic age and cellular senescence markers [1]. Scientists found that classical monocytes and naive B and T cells had lower transcriptomic age in moderate COVID-19 cases [1]. Human embryo cells showed signs of transcriptomic rejuvenation during morulae and blastocyst stages [1].
DNA Methylation Clocks and Epigenetic Biomarkers
DNA methylation patterns change predictably as we age, making them excellent biological timekeepers. Scientists have created more sophisticated “epigenetic clocks”:
We used first-generation clocks (Horvath’s multi-tissue clock and Hannum’s clock) to predict chronological age [27]
Second-generation clocks (PhenoAge and GrimAge) use mortality and morbidity data to better reflect biological aging [5]
These clocks predict chronological age within a few years in humans [27]. People with faster epigenetic aging face higher risks of cardiovascular disease, cancer, and death from any cause [5]. Studies of epigenetic aging biomarkers’ heritability revealed links to pro-inflammatory signaling pathways [5].
Proteomics and Metabolomics in Senescent Cell Profiling
Mass spectrometry-based proteomics offers a new perspective on aging through the senescence-associated secretory phenotype (SASP). Recent proteomic profiling found between 441 and 1,693 secreted proteins from senescent cells, varying by senescence inducer [28]. Research also showed changes in almost 40% of the mitochondrial proteome during cellular senescence [29].
Metabolomics studies small molecules in the body and gives great insights into aging processes. High-throughput nuclear magnetic resonance (NMR) analysis and machine learning algorithms have expanded this field to population-scale research [30]. A comprehensive study identified 54 aging-related metabolomic biomarkers from 250,341 people that predict death from any cause [30].
Resources for Further Research:
Limitations of Current Anti-Aging Strategies
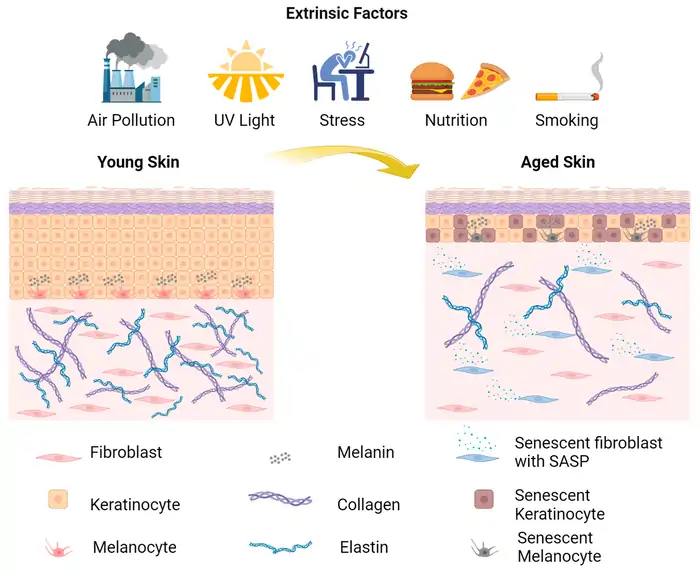
Image Source: MDPI
Anti-aging interventions show promise, but major obstacles limit their use in clinical settings. Scientists must address several challenges before these treatments become widely available.
Senolytics and Their Off-Target Effects
Senolytic drugs, which target and remove senescent cells, come with notable toxic effects. Patients using dasatinib experience fluid retention and myelosuppression. Navitoclax causes severe thrombocytopenia in 29 of 55 lymphoma patients [31]. The drug also harms osteoblasts and bone marrow progenitor cells through mechanisms we don’t yet understand, which leads to major bone mass reduction [31].
Toxicity isn’t the only concern. These drugs might interfere with helpful senescence functions. Research shows senescent fibroblasts help wounds heal faster by secreting platelet-derived growth factor AA in mice [31]. These cells also aid cardiac repair after heart attacks [31]. Random removal of senescent cells could limit these protective effects.
Challenges in Telomerase Activation Therapies
Treatments targeting telomerase face several key limitations. These therapies take too long to fight cancer because they depend on telomeres getting shorter with each cell division [6]. This delay gives cancer cells time to develop resistance [6].
The effectiveness of telomerase inhibitors remains limited because even minimal telomerase activity maintains short telomeres and helps tumors grow [6]. Clinical trials of imetelstat showed it caused dangerous side effects like thrombocytopenia and neutropenia, often forcing doctors to stop treatment [6].
Activating telomerase brings its own risks, mainly cancer concerns. Long-term telomerase suppression might harm fast-growing cells that need telomerase to survive [32].
Incomplete Reversal of Epigenetic Aging
Epigenetic age reversal looks promising but doesn’t deliver complete results. Benefits tend to fade after treatment stops, leaving only partial improvements. Research shows effects diminished after 6 months, keeping only 1.5 years of improvement [33].
Some measures show better lasting effects. GrimAge clocks maintained about 2.1 years of predicted lifespan improvement even after treatment ended [33].
Standardization poses another big challenge. Anti-aging treatment research lacks consistent ways to measure results, which makes it hard to determine what works [34]. Most treatments focus on single pathways while aging affects many connected systems [34].
Resources:
Aging Cell Journal
The Cellular Senescence Network
National Institute on Aging
Conclusion
Research into cellular aging has given us fascinating lessons about our biological timeline. Scientists have uncovered how our cells change from early-life protection through mid-life transitions to late-life decay. Young cells show us how telomerase activity, DNA repair efficiency, and redox balance work together. These processes are the foundations of longevity.
Mid-life brings important molecular changes. NAD+ levels start dropping and DNA damage increases. These changes lead cells to enter senescence – a crucial milestone where they stop dividing. Senescent cells release inflammatory compounds that affect nearby tissues. Late-life cellular breakdown shows through severe mitochondrial problems, protein clumping, and depleted stem cells. These issues directly connect to age-related diseases.
New tools like single-cell transcriptomics and epigenetic clocks have changed our understanding of aging mechanisms completely. Current anti-aging treatments don’t deal very well with certain challenges, especially when you have senolytic drugs and telomerase therapies. Yet this growing knowledge points to better treatments in the future.
Scientists are learning about targeted ways to keep cells healthy without unwanted side effects. Their research suggests that combining different approaches might work better than focusing on just one pathway. Looking at both energy metabolism and protein quality control could provide more complete solutions.
Recommended Resources:
National Institute on Aging (www.nia.nih.gov)
The American Federation for Aging Research (www.afar.org)
Nature Aging Journal (www.nature.com/nataging)
Aging Cell Journal (onlinelibrary.wiley.com/journal/14749726)
FAQs
What are the key molecular changes that occur during cellular aging?
Cellular aging involves several key molecular changes, including a decline in NAD+ levels, accumulation of DNA damage, epigenetic alterations, and the onset of cellular senescence. These changes lead to decreased DNA repair efficiency, mitochondrial dysfunction, and the activation of inflammatory pathways.
How do young cells maintain their health and longevity?
Young cells maintain their health through high telomerase activity, efficient DNA repair mechanisms, and optimal redox balance. They also have lower levels of oxidative damage due to more efficient mitochondrial function and robust antioxidant defense systems.
What is cellular senescence and how does it contribute to aging?
Cellular senescence is a state of permanent cell cycle arrest characterized by specific markers like p16INK4a and p21CIP1. Senescent cells develop a senescence-associated secretory phenotype (SASP), releasing inflammatory factors that can affect neighboring cells and contribute to chronic, low-grade inflammation associated with aging.
How are scientists studying cellular aging at the molecular level?
Scientists use advanced techniques like single-cell transcriptomics, DNA methylation clocks, and proteomics to study cellular aging. These methods allow researchers to analyze gene expression in individual cells, track epigenetic changes, and profile the proteins secreted by senescent cells.
What are some limitations of current anti-aging strategies?
Current anti-aging strategies face several challenges. Senolytic drugs, which target senescent cells, can have off-target effects and may disrupt beneficial senescence functions. Telomerase-targeting therapies struggle with efficacy and potential cancer promotion. Epigenetic age reversal treatments often show incomplete or temporary effects, highlighting the need for more comprehensive approaches to address multiple aging pathways simultaneously.
References
[1] – https://www.nature.com/articles/s42003-024-07094-5
[2] – https://pmc.ncbi.nlm.nih.gov/articles/PMC9784880/
[3] – https://pmc.ncbi.nlm.nih.gov/articles/PMC9909609/
[4] – https://pmc.ncbi.nlm.nih.gov/articles/PMC4911819/
[5] – https://pmc.ncbi.nlm.nih.gov/articles/PMC5940111/
[6] – https://pmc.ncbi.nlm.nih.gov/articles/PMC7678952/
[7] – https://www.nature.com/articles/npjamd201617
[8] – https://pmc.ncbi.nlm.nih.gov/articles/PMC7442590/
[9] – https://www.nature.com/articles/ncomms5211
[10] – https://pmc.ncbi.nlm.nih.gov/articles/PMC5088772/
[11] – https://elifesciences.org/articles/62852
[12] – https://www.nature.com/articles/s41392-022-01211-8
[13] – https://inflammregen.biomedcentral.com/articles/10.1186/s41232-022-00197-8
[14] – https://www.cellsignal.com/science-resources/overview-of-cellular-senescence?srsltid=AfmBOoqJAAaQkVPQHP9SLI57uofavrVfNZfJOL8wIKat_UbmO05KwUgw
[15] – https://pmc.ncbi.nlm.nih.gov/articles/PMC8698401/
[16] – https://pmc.ncbi.nlm.nih.gov/articles/PMC4166495/
[17] – https://www.nad.com/news/agings-vicious-cycle-senescence-inflammation-and-nad-depletion
[18] – https://www.aging-us.com/article/101617/text
[19] – https://www.jci.org/articles/view/64125
[20] – https://pmc.ncbi.nlm.nih.gov/articles/PMC9246372/
[21] – https://pmc.ncbi.nlm.nih.gov/articles/PMC5514379/
[22] – https://pubmed.ncbi.nlm.nih.gov/36778589/
[23] – https://www.pnas.org/doi/10.1073/pnas.0708931105
[24] – https://pmc.ncbi.nlm.nih.gov/articles/PMC3080271/
[25] – https://pmc.ncbi.nlm.nih.gov/articles/PMC4160113/
[26] – https://agmr.hapres.com/htmls/AGMR_1412_Detail.html
[27] – https://pmc.ncbi.nlm.nih.gov/articles/PMC6520108/
[28] – https://journals.plos.org/plosbiology/article?id=10.1371/journal.pbio.3000599
[29] – https://pmc.ncbi.nlm.nih.gov/articles/PMC10272782/
[30] – https://www.nature.com/articles/s41467-024-52310-9
[31] – https://academic.oup.com/ooim/article/4/1/iqad004/7151082
[32] – https://pmc.ncbi.nlm.nih.gov/articles/PMC4962009/
[33] – https://pmc.ncbi.nlm.nih.gov/articles/PMC6826138/
[34] – https://www.annualreviews.org/content/journals/10.1146/annurev-bioeng-120122-123054?crawler=true&mimetype=application/pdf